All Science Fair Projects
1000 science fair projects with complete instructions, organic vs. inorganic fertilizers.
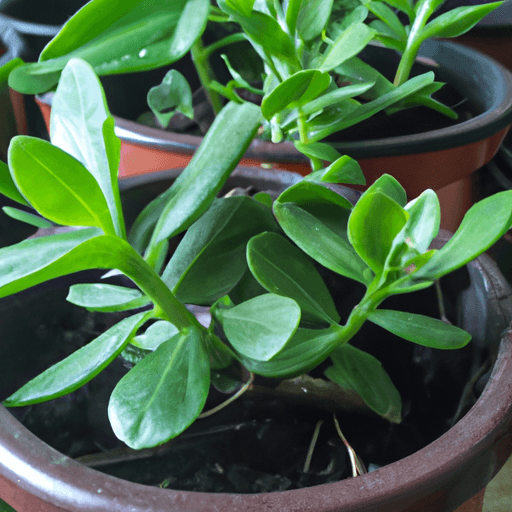

Method & Materials
Why do this project, also consider, full project details, related video.
Related Science Fair Project Ideas
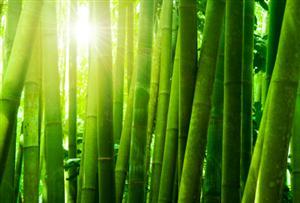
Plants can’t tell the difference: Understanding the pros and cons of organic and inorganic hydroponic fertilizers

- February 16, 2022
- General News
Inorganic fertilizers have a bad rep.
Often called “synthetic,” “chemical” and “artificial,” many believe inorganic fertilizers are full of dangerous ingredients that are harmful to humans and the environment.
This perception has driven many growers to choose organic fertilizers instead. Many view organic fertilizers as safer, cleaner and better for the environment. Some may believe that if the fertilizer is organic, they can label their plants organic too.
But the truth isn’t so black and white.
There’s no evidence that plants grown with inorganic fertilizers are unsafe for human consumption. While some inorganic fertilizers are synthetically produced, many are sourced from high-quality raw minerals naturally found in the earth. And the stories we hear about inorganic fertilizers damaging the environment are mostly traced back to traditional farming—a completely different production system from hydroponics.
Organic fertilizers certainly have their benefits, but those benefits can be harder to realize in hydroponic systems.
Neither organic nor inorganic fertilizers are inherently good or bad. There are pros and cons to each. Both have a place in hydroponics, and combining them can provide the best of both worlds. That’s what this white paper will address. First, we’ll explain the scientific differences between the different types of fertilizers and what those differences mean when it comes to regulations and the marketing of crops fertilized by them. Then we’ll discuss the advantages and challenges of using each. Finally, we’ll cover why growers don’t need to choose one over the other but can incorporate both for successful hydroponic production.
Defining the differences
Before we discuss the differences between the different types of fertilizers, let’s first define fertilizer.
Fertilizer is any material that supplies one or more nutrients essential to plant growth. The material can be organic or inorganic and sourced from natural material or made synthetically. 1 It can contain one of the three primary macronutrients—nitrogen (N), phosphorus (P) and potassium (K)—as well as secondary macronutrients, micronutrients or combinations of the above. Fertilizers can be applied directly to the plant roots or to the leaves (via foliar spray), or they can be applied indirectly through soil irrigation.
So what’s the difference between organic and inorganic fertilizers? In short, carbon (C). In addition to containing at least one mineral plant nutrient, organic fertilizers must contain carbon (as well as hydrogen and oxygen, which are also present in many inorganic fertilizer compounds). 2 This means they are usually derived from the byproducts or remains of an organism.

Inorganic fertilizers, on the other hand, don’t have carbon as an essential component of their makeup. They can be mineral, synthetic or chemical, with each differing in the degree of processing they undergo.
Mineral fertilizers can be manufactured or sourced from natural materials, such as rock or mineral salts, which are processed for plant absorption. Aside from nitrogenous fertilizers, they are usually purified minerals. 3
Phosphorus fertilizer is one example. The raw material, rock phosphate, is often used to manufacture it. But because the phosphorus availability in it is low, it’s usually treated in an electric furnace or with acid and gypsum to create orthophosphoric acid, the phosphate form plants can absorb. 4 The potassium in the fertilizer goes through a similar process. Minerals with potassium are mined and purified with dry and slurry processes, resulting in potassium chloride. Potassium chloride can then be treated with sulfuric acid at a high temperature to create potassium sulfate. 5
Synthetic fertilizers contain inorganic compounds usually derived from byproducts of the petroleum industry. For example, the fertilizer anhydrous ammonia is created by the Haber-Bosch process, where atmospheric nitrogen is combined with hydrogen at a high temperature and pressure. Steam reforming of natural gas can serve as the source of hydrogen for the process.6
Chemical fertilizers, on the other hand, are man-made fertilizers produced through chemical reactions. Urea can be considered a chemical fertilizer because it is developed from a process whereby ammonia and carbon dioxide react to create ammonium carbamate, which is then dehydrated to urea. 7
It’s important to remember that all fertilizers—both organic and inorganic—technically contain chemicals. The very nutrients plants need to survive and thrive are in fact chemical elements.
Organic fertilizer versus organic labeling
Now that we’ve covered the differences between organic and inorganic fertilizers, we need to clarify what it actually means to label a crop organic.
In the US, Canada, the European Union and many other countries, you can’t label fruits and vegetables, packaged food or other goods “organic” unless they’re certified through the governing body’s organic program. In the US, certification falls under the National Organic Program (NOP) of the US Department of Agriculture (USDA). 8 It’s illegal for any produce or goods to bear the USDA Organic Seal or use the word “organic” on its packaging without being reviewed and approved by a USDA-accredited certifying agent. 9
To receive organic certification, growers must follow production methods approved by their country or state. In the US, one of those practices includes using only organic fertilizers. 10 While there are a few exceptions, most inorganic fertilizers cannot be used in organic production. Those permitted are listed in the National List of Allowed and Prohibited Substances, which can be accessed via the USDA website: https://www.ams.usda.gov/rules-regulations/national-list-allowed-and-prohibited-substances.
While organic growers can use raw forms of organic fertilizer, such as manure, they can also check the Organic Materials Review Institute (OMRI) for fertilizers approved for organic production. 11 California growers can also check the state’s Organic Input Material (OIM) Program. 12 Products with the OMRI or OIM seals can be used on certified-organic acres and operations.
It must be stressed that even if a grower uses only organic fertilizers, they cannot sell their crops as organic without certification.

Can Cannabis be organic?
In the US, under the current USDA NOP standards, cannabis cannot be certified organic because it is still considered a Schedule I drug, and its production is illegal under federal law. Only hemp and hemp derived products such as CBD oil can be certified organic, since the 2018 Farm Bill permits hemp production.
However, states where cannabis is legal may have or be developing their own organic certification standards specifically for the crop. As of 2021, California’s Department of Food and Agriculture (CDFA) was in the process of creating a statewide certification program for cannabis that will be comparable to NOP standards. 13 Growers should check with their state’s or country’s agricultural department to learn if they can organically certify their cannabis in the state.

In the meantime, the Cannabis Certification Council (CCC) has developed its own standards and certification criteria under its Organically Grown Cannabis Certification Program. The program has adopted USDA organic regulations where practicable. Cannabis growers can seek certification from organic certifying agencies that have been accredited by the CCC. 14 Additional certifications are available through other organizations, which you can learn about at whatsinmyweed.org.
What does it really mean when the label says “organic”?
If you’ve shopped for organic products, you’ve probably noticed the labeling isn’t consistent. Some products have the USDA Organic Seal, while others may just say “made with organic.” So what’s the difference? The USDA NOP has four labeling categories for organic products. Here’s what they mean:
100% organic: One hundred percent of the ingredients are certified organic and the label may include the USDA Organic Seal on the primary display panel— the portion of the panel consumers are most likely to see.
Organic: The product and ingredients are certified organic and can use the USDA Organic Seal. However, non-organic ingredients permitted on the National List of Allowed and Prohibited Substances may be used in the product, provided they don’t make up more than five percent of the combined total ingredients.
Made with organic: At least seventy percent of the product contains certified organic ingredients. The USDA Organic Seal can’t be used and organic claims on the label must be specific: for example, “made with organic oats” instead of “made with organic ingredients.” Up to three ingredients or ingredient categories can be represented as organic. Remaining ingredients don’t need to be organic but must be non-GMO, and any non-ag products must be permitted by the National List of Allowed and Prohibited Substances, such as baking soda in baked goods.
Plants don't care about the source of nutrients
Despite the scientific and regulatory differences between organic and inorganic fertilizers, plants don’t care where their nutrients come from. In fact, at the level of essential plant nutrients, they can’t tell the difference between organic and inorganic fertilizers. That’s because they’re only capable of absorbing essential nutrients in certain forms. Figure 1 shows which chemical forms of each nutrient are commonly taken up by plants.
For example, plants can only absorb nitrogen as nitrate (NO3-) ions or ammonium (NH4 +) ions—both of which are inorganic forms. When a plant is fertilized with manure or compost where nitrogen is still in its organic form, it’s unavailable to the plant until it is converted into inorganic forms through a process called mineralization. 15

Microbes are necessary for organic fertilization
For mineralization to occur, microorganisms need to be present. Beneficial microbes are responsible for breaking down the organic forms of essential nutrients into their inorganic, plant-usable forms.
In traditional farming and production systems where plants grow in soil, those microorganisms are already present. But hydroponic systems don’t have enough microbes to mineralize nutrients into inorganic forms. Unless the organic fertilizer is already water-soluble and microbially pre-processed before it’s incorporated with the hydroponic solution, growers will need to apply a root inoculant to establish microbial colonies. 16
Inorganic fertilizers bypass the mineralization process because their nutrients are already in plant-usable forms. Most of these fertilizers are water-soluble, and plants can absorb their nutrients immediately.
Organic fertilizers pay off in soil
Because organic fertilizers require microbes to convert their nutrients into plant-absorbable forms, most of the benefits associated with organic fertilizers apply to soil-based growing systems.
One of the biggest benefits of applying organic fertilizers to soil is that they contribute to soil health. There’s evidence that applying manure with high carbon content increases soil organic matter, enriching the soil with nutrients. Adding organic matter like manure also helps reduce soil compaction, improve soil aggregation, and increase the soil’s water infiltration and water-holding capacities. 17
The contribution of organic matter also tends to give the soil pH a higher buffering capacity.18 The pH impacts nutrient availability, so if it’s not in the optimal range, plants may not get the nutrients they need (see Figure 2).
Another benefit is the fact that the nutrients in organic fertilizers are not immediately available to plants. The slow release of essential nutrients from mineralization means the plants can be fertilized throughout different growth stages. It also helps prevent salt damage to crops and reduces the odds of losing nutrients to the environment through leaching, erosion, denitrification and volatilization. 19
But these benefits don’t transfer to hydroponic systems. Without soil, plants might not get the microbial benefits of using organic fertilizers. In soil, organic fertilizers support a thriving microbial community that helps prevent disease, as beneficial microorganisms outcompete pathogens for resources.20 But hydroponic growers need to use microbial inoculants to reap those benefits. The inoculants can colonize the rhizosphere even in soilless conditions, but they won’t appear on their own; they have to be applied.

Inoculants aren’t the only supplement hydroponic growers need to apply. The organic matter that is so beneficial to soil tends to cause problems in hydroponics. Growers have reported organic fertilizers creating a sludge that clogs irrigation lines and emitters, 21 requiring the use of line cleaners to clear it out.
Because organic fertilizers often don’t completely decompose in hydroponic systems, phytotoxic compounds can accumulate. Dissolved oxygen also declines, and the combination can result in inhibited plant growth. 22 Moreover, fertilizers containing animalderived sources tend to go rancid in recirculating systems. 23
Finally, unlike the stability that organic fertilizers bring to soil pH, in hydroponic systems they tend to cause the pH to fluctuate. 24
Instant absorption with inorganic fertilizers
Inorganic fertilizers do not create these problems in hydroponic systems.
As we discussed earlier, plants can only take up nutrients in inorganic forms. So when hydroponic growers use inorganic fertilizers, they don’t have to add any microbial inoculants for nutrient conversion. Plants can absorb the essential nutrients in inorganic fertilizers immediately. Because hydroponics are a controlled environment where there’s no risk of nutrients being lost, there’s no benefit to the slow release of nutrients typical of organic fertilizers.
Inorganic fertilizers also don’t require as much pH management. In an experiment comparing an inorganic fertilizer with an organic fertilizer applied to hydroponically grown lettuce, the organic fertilizer caused wild pH swings. The pH often increased above 8, making nutrients unavailable to the plants. By contrast, the inorganic fertilizer required less pH adjusting, likely because of the pH buffer in the formulation.
The study also found that the inorganically fertilized lettuce had significantly higher fresh weight compared with the organically fertilized lettuce. Adjusting the pH of the nutrient solution of the inorganically fertilized lettuce did not significantly affect the fresh weight. However, it was necessary to adjust the pH in the nutrient solution of the organically fertilized lettuce to obtain higher fresh weight. Based on these results, the researchers advise using inorganic fertilizer for increased yields and a cleaner hydroponic system with less pH maintenance. 25
Thanks to the absence of organic matter, hydroponic growers also don’t have to worry about inorganic fertilizers going rancid or clogging the system.
For all these reasons, unless a grower is certified organic, or plans to become certified, there’s no reason why they shouldn’t consider including inorganic fertilizers in their hydroponic systems.

Why inorganic fertilizers got a bad name
Given the benefits of inorganic fertilizers in hydroponic systems, it’s natural to wonder why they have such a negative connotation attached to them. Again, it goes back to the differences between soil and soilless production systems.
One argument often made against inorganic fertilizers is that they pollute the environment. It’s true that the dead zone in the Gulf of Mexico 26 and harmful algal blooms that plague freshwater bodies like Lake Erie are caused by nitrogen and phosphorus fertilizer runoff, respectively, from farm fields. But that doesn’t mean that inorganic fertilizers are solely to blame. The Ohio Lake Erie Phosphorus Task Force, charged with identifying and evaluating the contributions of dissolved reactive phosphorus in Lake Erie, found a lack of evidence to differentiate the relative contributions of inorganic and organic fertilizers—in this case, commercial fertilizers versus manure. But it noted that commercial fertilizer use outweighed manure application and biosolids by a factor of two to one. 27

In other words, inorganic fertilizers may be blamed more because they are used more.
Part of the reason for nutrient runoff from farm fields is the amount of fertilizer applied. One study found that about one third of annual nitrogen fertilizer applications made to US cornfields is just to compensate for the long-term loss of soil fertility caused by soil erosion and organic matter loss.28 Excessive fertilization, whether of organic or inorganic fertilizers, increases the risk of environmental pollution, and it’s why farmers are encouraged to match fertilizer needs to crop uptake. 29
Hydroponics, by contrast, uses significantly less fertilizer—anywhere from 55 to 85 percent less than traditional farming, depending on the hydroponic system (see Figure 3). And, unlike traditional farmers, hydroponic growers can control what happens to their nutrient wastewater and prevent environmental harm by properly recycling or purifying it. 30
There is also concern over the impact of inorganic fertilizers on human health. Some of it is due to the presence of heavy metals in some inorganic fertilizers. For example, cadmium can be found in fertilizers made from rock phosphate. However, scientific assessments show that trace amounts of cadmium in phosphorus fertilizers are safe and pose no risk to the general public or to the farmers who use them. 31
The Environmental Protection Agency (EPA) has standards that limit the levels of heavy metals and other toxic compounds present in fertilizers made from hazardous waste. 32 The EPA believes that as long as they are properly manufactured and applied, and can safely and effectively substitute for virgin raw materials, some wastes can be used beneficially in fertilizers. However, the agency notes that a relatively small percentage of fertilizers are made from industrial waste, and those that are hazardous are only used in a small portion of those fertilizers. 33
Other concerns stem from the idea that organic foods are healthier than conventionally grown foods. Again, it’s important to remember that organically fertilizing a plant does not mean it is organically produced.
The evidence surrounding this belief is mixed. For instance, one meta-analysis looked at 237 studies and found no consistent differences between the vitamin content of organic and conventional foods. Phosphorus was the only nutrient higher in organic produce. Given that few people have a phosphorus deficiency, the researchers said this has little clinical significance. 34

However, another meta-analysis based on 343 peer-reviewed publications came to different conclusions. Researchers found that the concentration of antioxidants was significantly higher, and the concentration of cadmium significantly lower, in organic crops. They cite evidence that these results are connected to specific agronomic practices used in organic production, such as the absence of mineral and phosphorus fertilizers. 35
Other studies only see a difference in nitrate concentrations. One survey measured nitrogen in vegetables available in US retail stores and found most organic vegetables had lower nitrate concentrations than their conventional counterparts. 36 A nine-year greenhouse study on edible plants—including tomatoes, beets, carrots and chard— that compared organic fertilizers with inorganic ones found the organically fertilized plants had lower nitrate concentration in their edible parts. 37
There are other health benefits of organic food, such as less pesticide residue. 38 But that goes back to the pesticide-free production methods of organic farming—not whether the plant was grown with organic or inorganic fertilizer.
Maximize the benefits with both
Unless you’re certified organic, there’s no need to swear off inorganic fertilizers. As we’ve discussed, they’re easier to work with in hydroponic systems and provide plants the nutrients they need in the forms they can absorb for instant uptake.
But using inorganic fertilizers doesn’t mean you need to avoid organic fertilizers and the benefits they offer. Instead, why not use both?
There are fertilizers that contain both high-quality inorganic minerals to provide plants with their basic nutrient needs and organic compounds to fill in dietary gaps and provide additional benefits.
Here are some tips on what to look for to ensure the nutrient products you choose benefit your hydroponic grow operation:
- Make sure they are formulated for hydroponics: Using greenhouse-grade fertilizers is crucial to avoiding clogs in pumps and irrigation lines. Organic fertilizer products must be soluble and not contain filler material designed for soil. Moreover, hydroponic fertilizer products may have higher calcium and magnesium and contain micronutrients, unlike most fertilizers used in fields. 39
- Note the mineral content: A base-nutrient fertilizer should contain all the essential plant nutrients at levels that meet plants’ nutritional requirements. Keep in mind that if a fertilizer provides too little or too much of any nutrient, the plant will suffer a deficiency or possibly toxicity. Too little obviously means the plant isn’t getting enough of that essential nutrient, but overfeeding can cause the nutrient to lock out or inhibit the uptake of one or more other nutrients (see Figure 4).

- Research for organic compounds: What natural ingredients are in the product? More importantly, what do they do? Look for sources that are known to improve crop production. For example, kelp extract stimulates cellular growth, promotes plant strength, increases chlorophyll content and helps trigger early flowering and fruiting.
- Check for chelation: This may be in the form of chelated micronutrients or organic compounds such as humic acid. In either case, it will be stated on the label. By forming complexes that the plant roots can readily absorb, chelates help prevent nutrients from binding to the substrate or being lost as waste. They also extend the pH range of micronutrients to keep them absorbable for plants.
Emerald Harvest’s complete line of nutrient products is formulated to maximize plant growth in the best way possible. That means using the best raw materias for the job—sometimes organic substances, other times natural minerals, and often a mixture of both. The inorganic ingredients we use are high-quality and supplemented with organic compounds to give plants a broad palette of nutrient sources.. They are always clean and safe for you, the environment and most importantly for healthy, strong plants. Contact Emerald Harvest for information about our complete product line of premium nutrients. Emerald Harvest base nutrients and supplements help growers maximize the genetic potential of their crops. To set up an appointment with a representative, call 1.866.325.8235 or email [email protected].
- Kuntal Hati and Kalikinkar Bandyoopadhay, “Fertilizers (Mineral, Organic), Effect on Soil Physical Properties,” Encyclopedia of Agrophysics, Encyclopedia of Earth Sciences Series (2011): https://doi.org/10.1007/978-90-481-3585-1_20
- K. F. Isherwood, “Mineral Fertilizer Use and the Environment,” International Fertilizer Industry Association (2000): 7, https://issuu.com/rareearthminerals/docs/ifa-unep
- “Understanding phosphorus fertilizers” University of Minnesota Extension; accessed June 1, 2021, https://extension.umn.edu/phosphorus-and-potassium/understanding-phosphorus-fertilizers
- “Potassium fertilizers – Manufacturing process of Potassium fertilizers” Guichon Valves; accessed June 1, 2021, https://guichon-valves.com/faqs/potassium-fertilizers-manufacturing-process-of-potassium-fertilizers/
- Kazafy Sabry, “Synthetic Fertilizers; Role and Hazards,” (2015): https://doi.org/10.13140/RG.2.1.2395.3366
- “Urea Production and Manufacturing Process,” Independent Commodity Intelligence Services (2010); accessed July 10, 2021
- Some states may have their own State Organic Program, upon approval by the USDA National Organic Program, which allows them to oversee organic production and handling within the state.
- “About Organic Labeling,” Agricultural Marketing Service, US Department of Agriculture; accessed June 1, 2021 https://www.ams.usda.gov/rules-regulations/organic/labeling
- Pamela Coleman, “Guide for Organic Crop Producers,” National Center for Appropriate Technology, ATTRA Sustainable Agriculture (2012); accessed June 1, 2021, https://attra.ncat.org/product/guide-for-organic-crop-producers/
- OMRI is a third-party nonprofit accredited to ISO 17065 standards by the USDA Quality Assessment Division to review inputs, including fertilizers, intended for use in certificated organic production.
- OIM is run by the California Department of Food and Agriculture to register fertilizers used in organic crop and food production. OIM-labeled products comply with the California Fertilizing Materials Law and Regulations in addition to USDA NOP standards.
- “OCal Program,” California Department of Food and Agriculture (2021); accessed July 10, 2021; https://www.cdfa.ca.gov/calcannabis/ocal.html
- “#WhatsInMyWeed; Clean Cannabis Certifications” Cannabis Certification Council; accessed June 1, 2021, https://www.cannabiscertificationcouncil.org/blog/whatsinmyweed-clean-cannabis-certifications
- “Crop Plants Take Up (Absorb) Nutrients in Inorganic Form,” International Plant Nutrition Institute; accessed July 10, 2021, http://www.ipni.net/publication/envb.nsf/0/22E42BA77D7347E5852579E4006E886E/$FILE/09062-01-Enviro- Brief-01.pdf
- Makoto Shinohara, Chihiro Aoyama, Kazuki Fujiwara, Atsunori Watanabe, Hiromi Ohmori, Yoichi Uehara and Masao Takano, “Microbial mineralization of organic nitrogen into nitrate to allow the use of organic fertilizer in hydroponics,” Soil Science and Plant Nutrition, 57:2 (2011), 190-203, https://doi.org/10.1080/00380768.2011.554223
- Agustin Olivo, “How can animal manure help my soils be healthier and more productive?” University of Nebraska-Lincoln, Institute of Agriculture and Natural Resources, UNL Water; accessed June 1, 2021, https://water.unl.edu/article/manure-nutrient-management/how-can-animal-manure-help-my-soils-be-healthier-and-more
- “Soil pH” Soil Health – Guides for Educators, USDA NRCS 2014
- George Silva, “What organic fertilizers mean to plants and soil,” Michigan State University Extension; accessed June 1, 2021, https://www.canr.msu.edu/news/what_organic_fertilizers_mean_to_plants_and_soil
- Kimberly Williams and J.S. Nelson, “Challenges of using organic fertilizers in hydroponic production systems,” Acta Horticulturae, 1112 (2016): 365-370, https://doi.org/10.17660/ActaHortic.2016.1112.49
- David Kuack, “Organic vs. traditional hydroponic production: the top 3 differences,” Hort Americas; accessed June 1, 2021, https://hortamericas.com/blog/news/organic-vs-traditional-hydroponic-production-the-top-3-differences/#
- Kazuki Kano et al., “Effects of Organic Fertilizer on Bok Choy Growth and Quality in Hydroponic Cultures,”Agronomy 11 (2021): 491, https://doi.org/10.3390/agronomy11030491
- Kimberly Williams, Olivier Francescangeli and Jason Nelson, “Using Organic Fertilizers in Hydroponics and Recirculating Culture,” GPN magazine (2013); accessed June 1, 2021, https://gpnmag.com/article/using-organic-fertilizers-hydroponics-and-recirculating-culture/.
- Ryan Ronzoni and Neil Mattson, “A Guide to Home Hydroponics for Leafy Greens,” Cornell University (2020); accessed July 10, 2021, https://cpb-us-e1.wpmucdn.com/blogs.cornell.edu/dist/8/8824/files/2020/05/Guide-To-Home-Hydroponics-For-Leafy-Greens.pdf
- “Treating Nitrates,” USDA Natural Resources Conservation Service Iowa; accessed July 10, 2021, https://www.nrcs.usda.gov/wps/portal/nrcs/ia/technical/ecoscience/nutrient/nrcs142p2_008203/
- “Ohio Lake Erie Phosphorus Task Force Final Report Executive Summary,” Ohio Environmental Protection Agency (2010); accessed July 10, 2021, https://www.epa.state.oh.us/portals/35/lakeerie/ptaskforce/Task_Force_Final_Executive_Summary_April_2010.pdf
- W.S. Jang et al., “The Hidden Costs of Land Degradation in US Maize Agriculture,” Earth’s Future 9(2) (2021): https://doi.org/10.1029/2020EF001641
- “Source Water Protection Practices Bulletin Managing Agricultural Fertilizer Application to Prevent Contamination of Drinking Water,” United States Environmental Protection Agency (2001); accessed July 10, 2021, https://extension.usu.edu/waterquality/files-ou/Agriculture-and-Water-Quality/fertilizer.pdf
- “How Not to Dispose of Hydroponic Nutrients,” GAIACA Waste Revitalization (2020); accessed July 10, 2021, https://www.gaiaca.com/dispose-hydroponic-nutrients/
- Terry Roberts, “Cadmium and Phosphorus Fertilizers: The Issues and the Science,” Procedia Engineering 83 (2014): 52-59, https://doi.org/10.1016/j.proeng.2014.09.012
- To learn what heavy metals are present in a particular fertilizer product, and their amounts, visit aapfco.org/metals.
- “Agriculture Nutrient Management and Fertilizer,” United States Environmental Protection Agency; accessed June 1, 2021, https://www.epa.gov/agriculture/agriculture-nutrient-management-and-fertilizer
- Michelle Brandt, “Little evidence of health benefits from organic foods, study finds,” Stanford Medicine News Center (2012); accessed July 10, 2021, https://med.stanford.edu/news/all-news/2012/09/little-evidence-of-healthbenefits-from-organic-foods-study-finds.html
- Marcin Barański et al., “Higher antioxidant and lower cadmium concentrations and lower incidence of pesticide residues in organically grown crops: A systematic literature review and meta-analyses,” British Journal of Nutrition, 112(5) (2014): 794-811, https://doi.org/10.1017/S0007114514001366
- Maryuri Nunez de Gonzalez et al., “A Survey of Nitrate and Nitrite Concentrations in Conventional and Organic-Labeled Raw Vegetables at Retail,” Journal of Food Science, 80(5) (2015): https://doi.org/10.1111/1750-3841.12858
- J. F. Herencia et al., “Comparison between Organic and Mineral Fertilization for Soil Fertility Levels, Crop Macronutrient Concentrations, and Yield,” Agronomy Journal, 99 (2007): 973-983, https://doi.org/10.2134/agronj2006.0168
- Natalie Bumgarner and Robert Hochmuth, “Leafy Crop Production in Small-Scale Soilless and Hydroponic System,” University of Tennessee Institute of Agriculture (2019); accessed June 1, 2021; https://extension.tennessee.edu/publications/Documents/W844-B.pdf
Top Ten Projects
- Candle Race
- Home-Made Glue #1
- Soil Erosion
- Volcanic Gas
- Accelerate Rusting
- Vibrating Coin
- Mentos Soda Volcano
- Musical Bottles
- Human Battery Power
Latest Projects
- Sweet Erosion
- Your Planetary Age
- Exploding Ziploc
- Dehydrated Potato
- Homemade Windmill
Want to contribute?
Fertilizer & plants.
The purpose of this experiment is to find out which type of fertilizer, whether organic or inorganic, speeds the growth of plants.
Additional information
The hypothesis is that organic fertilizer will speed the growth of the plants due to the lack of toxins and pesticides found within the fertilizer.
Sponsored Links
Required materials.
- Six plastic cups
- Six bean plant seedlings, already germinated
- Six small plastic plates for drainage
- One bag of organic fertilizer of a particular brand
- One bag of inorganic fertilizer of the same brand as the organic
- Two to three gallons of distilled water
- Journal or logbook
Estimated Experiment Time
About three weeks.
Step-By-Step Procedure
- 1. Germinate bean plant seeds if you did not purchase them as seedlings.
- 2. Poke holes with scissors in the bottoms of the cups for adequate drainage.
- 3. When seeds are germinated or seedlings are purchased, fill three plastic cups with organic soil and the other three plastic cups with inorganic soil.
- 4. Plant seedlings in each cup.
- 5. Water every three days, or when the top of the soil is try to the touch. You will water with distilled water only to avoid possible contamination by any mineral deposits that are in the tap water.
- 6. Continue watering and growing plants for about three weeks.
You may need an adults help with cutting holes in the cups and planting the germinated seeds or seedlings.
Observation
Every three days, measure and record the height of the bean plants in millimeters. Record both the measurements for the bean pants potted in organic soil as well as the bean plants potted in the inorganic soil. When the project is complete, graph your results on a chart so you can easily determine which type of soil, whether it was organic or inorganic, produced the fastest plant growth.
Was the hypothesis correct? Did the organic soil speed the growth of the bean plants? If so, how much faster did the plants grow? Did the inorganic soil produce better results? If so, why? Why do you believe the hypothesis was either correct or incorrect? Be sure to answer any and all questions about the growth of the bean plants and the soils used to give a clear idea of which soil produced the fastest plant growth and why.
Take a moment to visit our table of Periodic Elements page where you can get an in-depth view of all the elements, complete with the industry first side-by-side element comparisons!
All Projects List
All categories.
home | about us | support | link to us | usage agreement | privacy policy | sitemap article resources -->
Copyright 2007, Sciencefairadventure.com. All Rights Reserved.
Thank you for visiting nature.com. You are using a browser version with limited support for CSS. To obtain the best experience, we recommend you use a more up to date browser (or turn off compatibility mode in Internet Explorer). In the meantime, to ensure continued support, we are displaying the site without styles and JavaScript.
- View all journals
- My Account Login
- Explore content
- About the journal
- Publish with us
- Sign up for alerts
- Open access
- Published: 22 April 2024
A global meta-analysis on the effects of organic and inorganic fertilization on grasslands and croplands
- Ting-Shuai Shi 1 ,
- Scott L. Collins ORCID: orcid.org/0000-0002-0193-2892 2 ,
- Kailiang Yu ORCID: orcid.org/0000-0003-0145-0356 3 ,
- Josep Peñuelas ORCID: orcid.org/0000-0002-7215-0150 4 , 5 ,
- Jordi Sardans ORCID: orcid.org/0000-0003-2478-0219 4 , 5 ,
- Hailing Li 1 &
- Jian-Sheng Ye ORCID: orcid.org/0000-0001-5335-7896 1
Nature Communications volume 15 , Article number: 3411 ( 2024 ) Cite this article
11k Accesses
7 Citations
3 Altmetric
Metrics details
- Agroecology
- Climate-change ecology
- Grassland ecology
A central role for nature-based solution is to identify optimal management practices to address environmental challenges, including carbon sequestration and biodiversity conservation. Inorganic fertilization increases plant aboveground biomass but often causes a tradeoff with plant diversity loss. It remains unclear, however, whether organic fertilization, as a potential nature-based solution, could alter this tradeoff by increasing aboveground biomass without plant diversity loss. Here we compile data from 537 experiments on organic and inorganic fertilization across grasslands and croplands worldwide to evaluate the responses of aboveground biomass, plant diversity, and soil organic carbon (SOC). Both organic and inorganic fertilization increase aboveground biomass by 56% and 42% relative to ambient, respectively. However, only inorganic fertilization decreases plant diversity, while organic fertilization increases plant diversity in grasslands with greater soil water content. Moreover, organic fertilization increases SOC in grasslands by 19% and 15% relative to ambient and inorganic fertilization, respectively. The positive effect of organic fertilization on SOC increases with increasing mean annual temperature in grasslands, a pattern not observed in croplands. Collectively, our findings highlight organic fertilization as a potential nature-based solution that can increase two ecosystem services of grasslands, forage production, and soil carbon storage, without a tradeoff in plant diversity loss.
Similar content being viewed by others
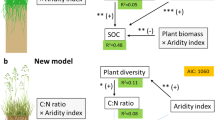

The positive effect of plant diversity on soil carbon depends on climate
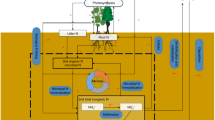
Negative to positive shifts in diversity effects on soil nitrogen over time
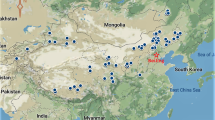
Nexus of grazing management with plant and soil properties in northern China grasslands
Introduction.
Global environmental change, such as increased atmospheric CO 2 and nitrogen enrichment, continues to threaten the ability of ecosystems to provide critical functions and services 1 . These challenges have motivated the development of nature-based solutions (e.g., organic fertilization and avoiding over-application of inorganic fertilizers) to mitigate the consequences of global environmental change through biodiversity conservation and increased carbon sequestration 2 , 3 . Yet, designing and implementing nature-based solutions that can address multiple sustainability goals require research targeted towards specific ecosystems and conservation objectives 4 .
Inorganic fertilizers have been widely applied to increase plant productivity in grassland and cropland ecosystems 5 , 6 . However, in grasslands, this management practice often alters plant community composition and diversity 6 , 7 . Indeed, most previous studies that have evaluated the effects of inorganic nitrogen 6 , 8 have found a general tradeoff occurs between increased aboveground biomass and lower plant diversity, with larger plant diversity losses as the amount and number of added nutrients increase 9 . Currently, a key knowledge gap is whether and how organic fertilization would potentially increase plant biomass without plant diversity loss. Addressing this question would require a better understanding of the underlying mechanisms of plant diversity loss following fertilization and the differences between organic and inorganic fertilizers in governing these mechanisms.
Three mechanisms have been proposed to explain the plant diversity loss in response to nutrient addition. The biomass-driven competition hypothesis postulates that greater aboveground biomass following inorganic fertilization increases interspecific competition for light, which excludes short-statured plant species 10 , 11 , 12 . The niche dimension hypothesis assumes that a larger number of limiting factors create more niche partitioning opportunities that facilitate species coexistence, whereas fertilization reduces the number of belowground niches 9 , 11 , 13 . Finally, the nitrogen detriment hypothesis proposes that long-term nitrogen fertilization may increase the susceptibility of plant species to stress agents 14 , 15 such as soil acidification 16 , ammonium toxicity 17 , and soil microbiome change 14 . The relative importance of these mechanisms has yet to be assessed across different environmental conditions such as vegetation properties, soil moisture, and nutrient availability. Indeed, soil water and nutrients influence plant growth and competition, and thus, both could regulate how plant diversity responds to fertilization 18 .
Few studies have assessed whether or not organic fertilization also leads to a potential tradeoff between aboveground biomass and plant diversity. Organic fertilizers derived from animal/plant residues and excrement supply organic matter and nutrients to soil, thus increasing soil organic carbon (SOC) storage, cation exchange, water-holding capacity, and microflora 19 , 20 while decreasing soil evaporative water loss. In contrast, inorganic fertilizers are chemically synthesized and may lack the capacity to improve certain soil properties, such as preventing soil acidification, as effectively as organic fertilizer can 16 , 21 . Thus, organic fertilization differs from inorganic fertilization in its influence on soil and vegetation conditions. These differences may result in divergent impacts on plant diversity between organic and inorganic fertilizer applications.
The light competition and niche dimension hypotheses both propose that increased aboveground biomass 22 and soil resource availability under organic fertilization should also reduce plant diversity. However, if nitrogen-driven soil acidification and ammonium toxicity were the main mechanisms causing plant diversity loss after nutrient addition, organic fertilization should not result in a decline in plant diversity. As such, organic fertilizers added to grasslands increase biomass and carbon storage without a tradeoff in plant diversity loss. To date, studies have observed that organic fertilization may decrease 16 , increase 23 , or have no significant effect on plant diversity 22 . However, the number of species in a community is also limited by soil pH 24 , moisture 18 and soil fertility 25 . Therefore, the plant diversity response to organic fertilization may depend on local conditions. Assessing the effect of organic fertilization on plant diversity and understanding the underlying mechanisms are thus vital to evaluate tradeoffs between biomass production, soil carbon storage, and plant diversity loss.
Another central role for nature-based solutions is to identify optimal management practices to increase carbon sequestration. A better understanding of carbon sequestration potential following fertilization would need a full assessment of aboveground and belowground responses. SOC, the largest carbon pool in terrestrial ecosystems 26 , 27 , plays a key role in maintaining soil fertility and improving agricultural productivity and sustainability 28 . However, the response of SOC under organic versus inorganic fertilization remains uncertain 29 , 30 because it may depend on local climate 31 , land-use types 32 and nutrient availability 33 .
To evaluate the potential for organic fertilizers to be used as a nature-based solution, a full assessment of its benefit is needed across land-use types that often receive additional nutrients to increase production, such as grasslands and croplands. Indeed, organic fertilization has been shown to increase SOC stocks by about 30% in both croplands and grasslands 34 , 35 . However, due to carbon storage differences between land-use types, the nutrient requirements for carbon sequestration may differ between grasslands and croplands 36 . Soil carbon storage in croplands is also affected by agricultural managements practices 37 , 38 such as fertilizer rate and tillage, which may lead to different SOC responses to organic fertilization than in grasslands. It remains unclear the degree to which global grasslands and croplands differ in carbon sequestration potential under comparable organic fertilization rates.
To assess the effects of organic versus inorganic fertilization on plant biomass, plant diversity, and SOC, we compiled >5000 pairs (ambient versus fertilization) of measurements from 537 experiments across grasslands and croplands worldwide (Supplementary Fig. 2 ). We focused on grasslands because a large number of fertilization experiments were conducted in this ecosystem 10 . Moreover, grasslands account for about 41% of the global land surface excluding Greenland and Antarctica. They store around 34% of the terrestrial carbon stock and provide a variety of ecosystem services including forage production, plant diversity conservation, and climate regulation 39 . Croplands are widespread globally, and most agricultural practices reduce soil carbon content 38 , 40 , thus organic fertilizers have the potential to increase SOC under agricultural management practices. We hypothesized that: (1) organic fertilization would increase aboveground biomass more than inorganic fertilization in grasslands, (2) if increased biomass production intensified competition for light, or fertilization reduced belowground niche partitioning, organic fertilization would also cause a decline in plant diversity in grasslands, and (3) if nitrogen detriment (e.g., acidification) was the main mechanism, organic fertilization would not cause plant diversity loss in grasslands. We also evaluated the effect of fertilization on biomass, plant diversity, and SOC across environmental gradients to determine under which conditions tradeoffs among the three ecosystem services would be minimized. Finally, we hypothesized that (4) organic fertilizer added to croplands would lead to comparable increases in SOC relative to grasslands.
Global mean responses to fertilization
Inorganic fertilization significantly increased aboveground biomass by 42% ( p < 0.001) relative to ambient conditions, accompanied by an 18% ( p < 0.001) decline in species richness and 6% ( p < 0.001) decline in evenness in semi-natural and natural grasslands (Fig. 1a–c ). In contrast, organic fertilization significantly increased aboveground biomass by 56% ( p < 0.001), without reducing plant diversity relative to ambient conditions (Fig. 1a–c ). Compared to inorganic fertilization, organic fertilization significantly increased both species richness and evenness by 10% ( p < 0.001), while maintaining greater aboveground biomass (Fig. 1a–c ). Organic fertilization also significantly increased SOC by 19% ( p < 0.001) and 15% ( p < 0.001) compared to ambient conditions and inorganic fertilization, respectively. Inorganic fertilization significantly increased SOC by 2% (Fig. 1d , p < 0.001). Thus, although both fertilizers increased aboveground biomass, organic fertilization increased SOC more than did inorganic fertilization while also increasing species richness.
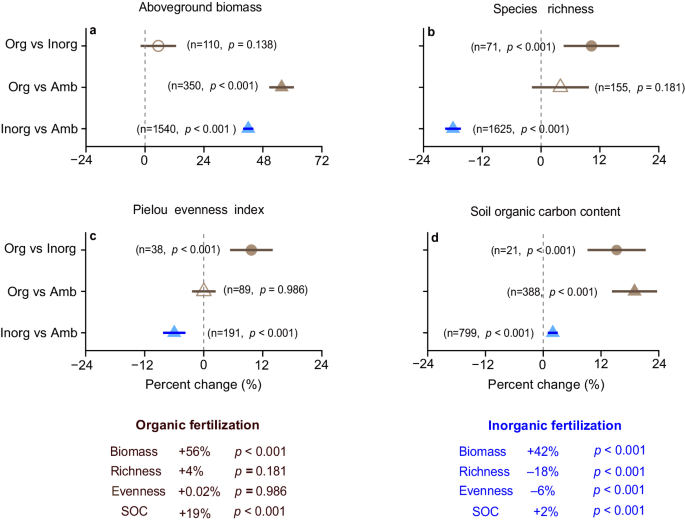
Percent changes in aboveground biomass ( a ), species richness ( b ), Pielou evenness index ( c ) and soil organic carbon (SOC) content ( d ) under organic and inorganic fertilization. Brown and blue triangles represent percent changes under organic and inorganic fertilization relative to ambient conditions, respectively. Brown circles represent percent changes under organic fertilization relative to inorganic fertilization in the same experiments. Filled symbols represent statistical significance when the 95% confidence intervals do not overlap with zero (two - tailed test, p < 0.05). The numbers in parentheses show the sample sizes. The sample sizes of SOC are the studies that measured both SOC and aboveground biomass or species richness ( d ). See Supplementary Table 2 for the value of percent changes and confidence intervals. Key: organic fertilization (Org), inorganic fertilization (Inorg), ambient conditions (Amb), aboveground biomass (Biomass), species richness (Richness) and Pielou evenness index (Evenness). Source data are provided as a Source Data file.
Fertilization responses across environmental gradients
Based on a multi-model inference procedure for selecting the set of best-fitting models (Supplementary Tables 4 – 8 ), we found that the effects of organic fertilization on aboveground biomass depended on climate and soil properties (Fig. 2a ). Grasslands with greater mean annual temperature (MAT), soil total nitrogen and fertilizer rate exhibited larger increases in aboveground biomass under organic fertilization ( p < 0.01, Fig. 2a–d , and Supplementary Table 9 ). Similarly, grasslands with greater soil bulk density (SBD), water content, and cation exchange capacity had larger increases in species richness under organic fertilization ( p < 0.05, Fig. 2e–h , and Supplementary, Table 9 ). Conversely, under inorganic fertilization, grasslands with greater nitrogen fertilizer rate, number of nutrients added, and soil water content (SWC) showed larger increases in aboveground biomass ( p < 0.05, Fig. 3a–d , and Supplementary, Table 10 ). However, increasing inorganic fertilizer rates in grasslands led to larger declines in species richness ( p < 0.001, Fig. 3e–g , and Supplementary Table 10 ).
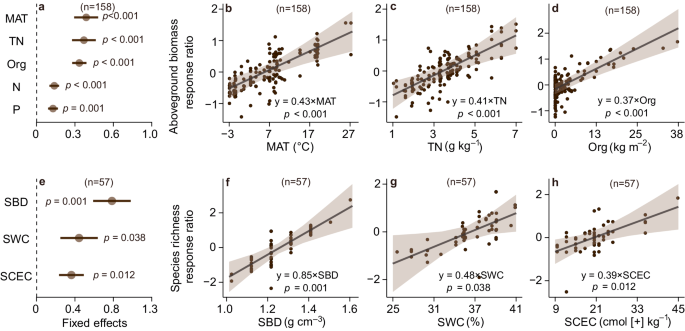
a , e Brown circles and error bars represent average parameter estimates (standardized regression coefficients) and the 95% confidence intervals in the linear mixed effects models (two – sided), respectively. The numbers in parentheses show the sample sizes. b – d , f – h The solid line in each panel shows model fit using partial regression for each environmental factor, and the shading around the fitted line represents the 95% confidence intervals (i.e., error bands represent slopes ± 95% confidence intervals). Equations in b – d and f – h show the values of standardized regression coefficients. The slopes of the partial regressions are the same as the fixed effects shown in Fig. 2 a , e . Complete model statistical results are presented in Supplementary Table 9 . Keys: MAT mean annual temperature, Org organic fertilizer amount added, TN soil total nitrogen, N nitrogen fertilizer rate, P phosphorus fertilizer rate, SBD soil bulk density, SCEC soil cation exchange capacity, SWC soil water content. The p values are calculated from two-tailed tests. Source data are provided as a Source Data file.
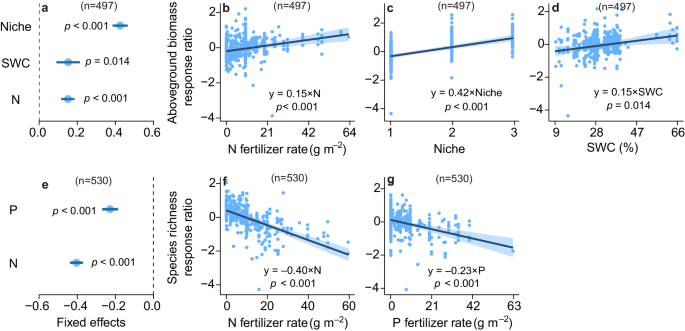
a , e The blue circles and error bars represent average parameter estimates and the 95% confidence intervals in the linear mixed effects models (two–sided), respectively. The numbers in parentheses show the sample sizes. b – d , f – g The solid line in each panel shows model fit using partial regression for each environmental factor, and the shading around the fitted line represents the 95% confidence intervals (i.e., error bands represent slopes ± 95% confidence intervals). Equations in b – d and f – g show the values of standardized regression coefficients. The slopes of the partial regressions are the same as the fixed effects shown in 3 a , e . Complete model statistical results are presented in Supplementary Table 10 . Keys: Niche number of nutrients added, N nitrogen fertilizer rate, P phosphorus fertilizer rate, SWC soil water content. See Fig. 2 for more details. Source data are provided as a Source Data file.
Relationship between biomass production and plant diversity
Our partial regression and structural equation model (SEM) showed only a weak negative link between the responses of biomass production and species richness under organic fertilization in grasslands (Fig. 4a and Supplementary Fig. 5a ). Both organic and inorganic fertilization increased aboveground biomass but only the latter decreased species richness (Fig. 4b and Supplementary Fig. 5b ). The SEM indicated that SWC had a positive link with the response of species richness under organic fertilization (Supplementary Fig. 5a ), which was consistent with the result of linear mixed effects models (Fig. 2g ).
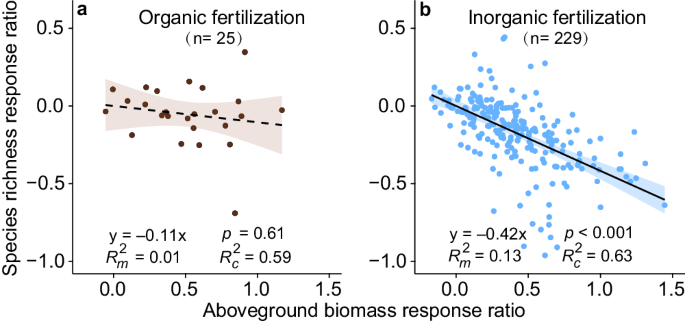
Field experiments that measured both aboveground biomass and species richness are used in the analyses. a Organic fertilization. b Inorganic fertilization. We use linear mixed effects models to evaluate the link between the response ratio (ln RR) of biomass and richness ( a , b ). The lines in ( a , b ) show model fit using partial regression for aboveground biomass, and the shading around the fitted line represents the 95% confidence intervals (i.e., error bands represent slopes ±95% confidence intervals). Solid and dashed lines denote statistically significant (two-sided p ≤ 0.05) and nonsignificant (two-sided p > 0.05) relationships, respectively. The slope of the regression between species richness and aboveground biomass is significantly greater under inorganic fertilization than under organic fertilization ( p slope difference = 0.023), according to the Standardized Major Axis Tests. Marginal R m 2 and conditional R c 2 indicate R 2 of fixed and random plus fixed effects ( a , b ), respectively. The numbers in parentheses show the sample sizes. Complete model statistical results are presented in Supplementary Table 11 . Source data are provided as a Source Data file.
SOC responses to organic fertilization in grasslands and croplands
In both grasslands and croplands, the effects of organic fertilization on SOC varied with climate, soil properties, and fertilizer rate (Fig. 5b ). Organic fertilization increased SOC more in croplands than in grasslands (32% versus 14% increases, respectively, Fig. 5a ) in areas with mean annual temperature (MAT) <15 °C. While in warmer regions, SOC increased more in grasslands than croplands (62% versus 34% increase, respectively, Fig. 5a ). After accounting for soil properties and organic fertilizer rate, the effect of organic fertilization on SOC increased significantly with MAT in grasslands ( p < 0.05, Fig. 5b, c ). These results remained consistent when we used a subset (about 40% of all studies) of the data that measured the quality (C:N ratios) of organic fertilizers (Supplementary Fig. S6 ). Therefore, under a future, warmer climate, applying organic fertilization to grasslands may result in a larger soil carbon sequestration potential than in croplands.
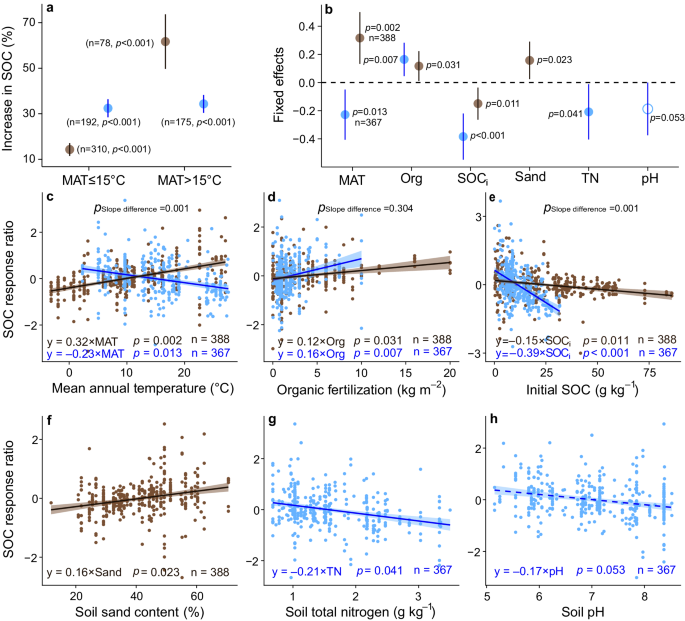
Blue and brown represent cropland and grassland, respectively. a Percent increase in SOC under organic fertilization in grasslands and croplands. b Effects of environmental factors on SOC responses under organic fertilization. b Circles and error bars represent average parameter estimates and 95% confidence intervals in the linear mixed effects models (two - sided), respectively. c – h The brown and blue lines in each panel show model fits using partial regression for each environmental factor, and the shading around the fitted lines represents the 95% confidence intervals (i.e., error bands represent slopes ±95% confidence intervals). The slopes of the partial regressions are the same as the fixed effects shown in b . Equations in c – h show the values of standardized regression coefficients. a – h The symbol n shows the sample sizes. c – h Complete model statistical results are presented in Supplementary Tables 6 and 9 . Keys: MAT mean annual temperature, Org organic fertilizer amount added, SOC i initial soil organic carbon, Sand: soil sand content, TN soil total nitrogen, pH soil pH, SBD soil bulk density. The p values are calculated from two-tailed tests. Source data are provided as a Source Data file.
As expected, the effect of organic fertilization on SOC increased with organic fertilizer rate in both grasslands and croplands ( p < 0.05, Fig. 5b, d ). Both the linear mixed effects model (Fig. 5b ) and the standardized major axis tests (Fig. 5d and Supplementary Table 13 ) showed equal SOC increases in grasslands and croplands under comparable organic fertilization rates. The effect of organic fertilization on SOC declined as initial/background SOC in both grasslands and croplands increased, and this pattern was stronger in croplands than in grasslands ( p < 0.05, Fig. 5b, e ). This result has two implications. First, it is not necessary to start with low initial organic carbon for organic fertilizer to increase SOC (Fig. 5e ). Second, under similar initial conditions, organic fertilization has a larger effect on SOC in grasslands than in croplands (Fig. 5b ).
Using a comprehensive dataset compiled from grassland fertilization experiments worldwide, we found that organic fertilization significantly increased biomass and SOC relative to ambient conditions, with no concomitant plant diversity loss (Fig. 1 ). Moreover, organic fertilization increased the plant diversity of native species in grasslands with greater soil water content (Fig. 2g ). Greater aboveground biomass would increase leaf area, transpiration and canopy interception, and decrease water infiltration 41 , 42 , potentially intensifying plant demand for soil water under fertilization. However, this mechanism may not occur in grasslands with greater soil water content, because water is rarely limiting in ecosystems with greater mean soil moisture. Organic fertilization increased SOC and cation exchange capacity, thus increasing water holding capacity 34 , 43 , 44 , and decreasing soil water evaporative loss. Therefore, organic fertilization would mitigate biomass-driven declines in soil water, thus avoiding plant diversity loss. Previous studies also supported this finding that mesic grasslands had greater plant species richness than arid grasslands 18 , 45 . Collectively, these findings support the significant link between soil water and plant diversity in many grasslands.
Our results from either organic or inorganic fertilization in grasslands do not support the niche dimension hypothesis, as the number of nutrients added was not selected in the best fitting models for species richness (Figs. 2e , 3e ). The niche dimension hypothesis predicted greater plant diversity loss with an increasing number of added nutrients 9 , 11 , 13 . One recent study of inorganic fertilization and water addition tested this hypothesis and found negligible support 10 . Organic fertilizers contain several macro- and micro-nutrients (Supplementary Data 1 ) but did not reduce plant diversity (Fig. 1 ), providing no support for niche dimension mechanism. Instead, the components of organic fertilizers would increase soil heterogeneity 46 , which may increase the number of micro-niches for plant species, thus reversing the niche dimension hypothesis.
According to the nitrogen detriment hypothesis, inorganic nitrogen increases susceptibility of plants to various stress agents, such as ammonium toxicity, soil acidification, and altered soil microbiome, all of which can lead to plant diversity loss 10 , 14 , 15 , 16 , 17 . We found that both nitrogen and phosphorus fertilizer rates decreased plant diversity (Fig. 3f–g ). As such, plant diversity loss under inorganic fertilization might not solely be related to greater nitrogen availability 47 . Indeed, many studies provide robust evidence that inorganic phosphorus addition leads to species loss 48 , 49 . We did not find a significant effect of organic fertilization rate on plant diversity (Supplementary Fig. 5a ). Organic fertilization improved the soil environment for plant growth by increasing nutrient availability, pH buffering, cation exchange, water holding capacity, and microbial community functioning 43 , 50 , 51 . Organic fertilizers need to be decomposed and thus may be less plant-available immediately following application 52 , 53 . Therefore, organic fertilizer releases nutrients slowly as it decomposes and may continuously provide multiple macro- and micro-nutrients for plant growth 43 , 54 . According to a subset (about 6% of all studies) of the data that measured both macro- and micro-nutrients (Supplementary Data 1 ), organic fertilizers supply nitrogen, phosphorus, potassium, magnesium, calcium, zinc, iron, etc. As a result, organic fertilizers increased plant biomass more than did inorganic fertilizers (56% ± 5% versus 42% ± 2%; Fig. 1 ). A previous study proposed that the average supply rate of the most limiting resource controlled plant diversity 55 . Organic fertilizers provide multiple nutrient resources and thus may avoid plant diversity decline. Collectively, organic fertilization increases soil fertility and aboveground biomass while limiting detrimental effects on plants and microbial diversity.
Organic fertilizers can serve as a nature-based solution to mitigate the tradeoff between increasing aboveground biomass and species loss in grasslands (Fig. 1 ). Studies showed that the increase in plant diversity under organic fertilization was due to improved soil fertility status, such as water holding capacity and nutrient availability 23 , 56 . Our finding clearly demonstrates that organic fertilization supports greater native plant diversity compared to inorganic fertilization. Thus, organic fertilization may also facilitate the restoration of degraded grasslands and abandoned croplands by increasing SOC, plant biomass, and plant diversity. However, studies have also reported an increase in exotic plant species with manure application 57 . Thus, we carefully selected and excluded organic fertilization experiments that included exotic plant seeds. As such, the plant diversity response to manure application was not caused by exotic species in our analyses. To avoid introducing exotic plant species and other side-effects, locally-sourced, noncontaminated manure or industrial organic fertilizers should be used as a nature-based solution.
We found that SOC response ratio under organic fertilization increased in grasslands but decreased in croplands under warmer mean annual temperature (Fig. 5c ). This difference may result from management practices, where croplands are typically tilled while grasslands are not. Tillage can disrupt soil aggregates and accelerate SOC decomposition by microorganisms 38 . Temperature is also an important factor driving SOC decomposition 58 , 59 . Tillage in warmer regions may increase SOC decomposition rate, thus leading to a smaller increase in SOC in croplands despite organic fertilization. By contrast, grasslands are rarely tilled. Organic fertilizer increases biomass production in grasslands, which may override the greater loss rate of SOC through decomposition as mean annual temperature increases 60 , 61 . Irrigation increases soil moisture, and thus affects SOC decomposition and carbon sequestration 62 . Croplands are usually irrigated, while grasslands are not. Warmer temperatures can accelerate soil moisture evaporation, which could slow SOC decomposition in grasslands but not necessarily in croplands. This result suggests that organic fertilization in grasslands may increase soil carbon sequestration potential in a future, warmer climate.
We found that the potential for soil carbon sequestration increased with organic fertilization rate in both grasslands and croplands (Fig. 5b ). Considering whole-system feedbacks, increased aboveground productivity following organic fertilization may support more livestock and produce more manure to fertilize grasslands, thus creating a positive feedback loop. However, croplands usually receive imported organic fertilizer from other locations 63 , potentially increasing transportation and application costs, which can reduce profit margins. We also tested the impact of organic fertilizer quality on SOC response 64 , 65 . Organic fertilizers with greater carbon:nitrogen ratios led to greater SOC increase in grasslands but less so in croplands (Supplementary Fig. 6 ), suggesting that low quality organic fertilizers may still promote carbon sequestration in grasslands. Thus, use of organic fertilization in grasslands might be more feasible than in croplands in terms of transportation costs and fertilizer quality.
Collectively, our results highlight the potential of organic fertilization to serve as a nature-based solution that improves grassland ecosystem functions and services, including carbon sequestration, and such potential may actually increase with climate warming in some regions. We found that organic fertilization increased aboveground biomass production and SOC without a tradeoff in plant diversity loss. By comparison, increased aboveground biomass production and soil carbon storage resulted in plant diversity loss under inorganic fertilization. As such, organic fertilization is favored over inorganic fertilization to improve grassland ecosystem functions and services. Organic fertilization increased plant diversity in grasslands with greater soil moisture. Moreover, organic fertilization may improve the soil environment for plant growth, and thus would avoid plant diversity loss caused by soil acidification and ammonium toxicity that is usually found under inorganic fertilization. Therefore, we argue that increasing the use of organic fertilizers would provide an important nature-based solution to increase productivity and soil carbon sequestration while conserving plant diversity.
Data collection
To compile data on the responses of plant biomass, plant diversity, and SOC to nutrient addition, we searched for peer-reviewed literature published before 30 October 2022 using the web of science and China National Knowledge Network resources. We used the following keywords: (resource addition OR resource availability OR nutrient addition OR nutrient availability OR nitrogen deposition OR nitrogen addition OR nitrogen enrichment OR phosphorus addition OR phosphorus enrichment OR potassium addition OR potassium enrichment OR organic fertilizer OR organic* OR manure* OR farmyard manure* OR pig manure OR cow manure OR horse manure OR sheep manure OR chicken manure OR wet compost) AND (species richness OR plant diversity OR biomass OR aboveground biomass OR AGB OR dry matter yield OR SOC OR soil organic carbon OR SOM OR soil organic matter OR SOC storage) AND (grassland OR meadow OR steppe OR prairie OR herbaceous OR annual OR cropland).
We used three criteria to select literature: (1) field experiments were conducted in semi-natural or natural grasslands, or croplands, and included both ambient and nutrient addition treatments; (2) the means, standard errors or standard deviations and sample sizes were reported; and (3) grassland studies reporting exotic plant species introduced by organic fertilization were excluded (Supplementary Fig. 1 ). Data were collected from tables in the main text or supporting information when available, or digitally extracted from figures using GetData Graph Digitizer software version 2.26 http://getdata-graph-digitizer.com/ .
Our search yielded 537 publications across all continents except Antarctica (Supplementary Fig. 2 ). In summary, we compiled 1540 pairs (ambient versus fertilization) of field measurements of aboveground biomass, 1625 pairs of species richness, 191 pairs of Pielou evenness index, and 799 pairs of SOC under inorganic fertilization. Under organic fertilization, we compiled 350 pairs of aboveground biomass, 155 pairs of species richness, 89 pairs of Pielou evenness index, 388 pairs of SOC in grasslands and 367 pairs of cropland SOC. Most field experiments in grasslands measured plant aboveground biomass and plant diversity during the peak growing season. In highly managed agroecosystems, only one or two crop species were maintained and thus it is not reasonable to analyze plant diversity. Therefore, we did not collect plant diversity data from cropland.
In our compiled dataset, inorganic/chemical fertilizer included urea (chemically synthesized from inorganic matters), ammonium nitrate, calcium nitrate, super-phosphate, ammonium phosphate dibasic, sodium dihydrogen phosphate, potassium sulphate and potassium chloride. None of the inorganic studies added liquid ammonia fertilizer. Organic fertilization included industrial organic fertilizer, livestock manure and compost. In 12% of field experiments, industrial organic fertilizers were applied, where organic matter was fermented and decomposed at high temperature, and thus any plant seeds within them were killed. The other 88% of experiments applied livestock manure or compost that was usually composted under high temperature and anaerobic environments, and then air-dried to kill plant seeds that may be contained in them. No exotic plant species were reported in all of the organic fertilization field experiments included in our meta-analysis. In 12% of the organic fertilization experiments, inorganic nitrogen, phosphorus, and potassium were also added. About 97% of the field experiments had a duration of <10 years in grasslands, while 74% of the experiments had a duration of >10 years in croplands.
Meta-analysis
We used the natural log-transformed response ratio (ln RR) to quantify the effect of nutrient fertilization on aboveground biomass, plant diversity, and SOC. The ln RR, also called “effect size”, was dimensionless and used to characterize the relative changes between treatment and control 66 .
Where \({\bar{{{\mbox{Y}}}}}_{{{{{\rm{t}}}}}}\) and \({\bar{{{\mbox{Y}}}}}_{{{{{\rm{c}}}}}}\) are sample mean values of the response variables (aboveground biomass, or plant diversity, or SOC) in the treatment group (t) and control group (c), respectively. In this study, we calculated two types of response ratio: (1) organic, inorganic fertilization relative to ambient condition (i.e., Org vs. Amb, Inorg vs. Amb) of all experiments, and (2) organic fertilization relative to inorganic fertilization (i.e., Org vs. Inorg) when conducted in the same experiments.
We used a hierarchical model with inverse variance weighting to summarize the response ratio (ln RR) from all individual studies, as this model was usually appropriate for biological experiments 66 , 67 . Because multiple treatments may share a single control, we added “ site ” as a random factor in the meta-analysis model to account for non-independence of observations collected from the same site 68 , 69 .
We used the “ rma.mv ” function in R “ metafor ” package version 4.4.0 to calculate the weighted mean response ratio (ln RR + +) and the 95% confidence intervals 70 . The 95% confidence intervals were generated by bootstrapping. When they did not overlap with zero, the treatment effects were considered statistically significant.
We applied the Egger’s test to examine publication bias 71 , and used the trim and fill approach to evaluate the impact of publicaton bias on the meta-anaysis results (Supplementary Table 1 and Fig. 3 ).
To ease interpretation, we transformed the weighted mean response ratio (ln RR + +) to percent change in the response variables by Eq. 2 :
Response of biomass, plant diversity and SOC across environment gradients
SOC response ratios related to potential moderators, including mean annual temperature (MAT), soil cation exchange capacity (SCEC), soil total nitrogen (TN), soil pH (pH), soil bulk density (SBD), initial soil organic carbon density, soil sand content (Sand) and soil water content (SWC) and fertilizer rates. We used species richness in this analysis because it had larger sample sizes than other plant diversity indices such as Pielou’s evenness (e.g., 155 versus 89 pairs in grasslands). MAT (°C) at each site was extracted from the WorldClim 2 datasets with 30 s spatial resolution 72 . SCEC (cmol [+] kg −1 ), total nitrogen (g kg −1 ), pH, bulk density (g cm −3 ), organic carbon density (kg m −3 ) and sand content (%) at 0 – 30 cm were extracted from the Soil Grid datasets with 30 s spatial resolution 73 . We used the ERA5-Land datasets of SWC (%) at 0 – 28 cm with 0.1° spatial resolution, with the mean values calculated during 1982 – 2022 74 .
We used linear mixed effects models to evaluate the response of biomass, plant diversity, and SOC to nutrient fertilization across environmental gradients, with study site as a random effect. We conducted linear mixed models in “lme4” package version 1.1-35.1 and “lmerTest” packages version 3.1.3. To select the set of environmental factors that significantly influenced the response of biomass, plant diversity and SOC to nutrient addition, we conducted a multi-model inference procedure based on the Akaike Information Criterion, using the dredge function in R “MuMIn” package version 1.47.5. To further strengthen the multi-model inference, we also conducted a random forest model to identify the significant environmental predictors of biomass and plant diversity. We used random forest models in the “randomForest” package version 4.7-1.1 to quantify the importance of each predictor, and then used the “rfPermute” package version 2.5.2 to assess the statistical significance of each predictor. The environmental factors selected by the best linear mixed effects model were also significant predictors identified by a random forest model (Supplementary Fig. 4 ).
We also employed structural equation model (SEM) to disentangle the direct and indirect effects of environmental factors and nutrient fertilization rate on the response ratio (ln RR) of biomass and species richness. We conducted SEM using the R “ lavaan ” package version 0.6-17, and evaluated goodness of the SEM model according to the criteria reported by ref. 75 .
To explore whether and where organic fertilizer added to grasslands led to greater increases in SOC than in croplands, we compared the slopes of the regression of SOC response ratio and environmental drivers (MAT, the amount of organic fertilization added, initial SOC, soil nutrients, soil texture, and pH) between grasslands and croplands, using the Standardized Major Axis Tests and Routines with ordinary least squares regression technique 76 .
Reporting summary
Further information on research design is available in the Nature Portfolio Reporting Summary linked to this article.
Data availability
All data used in this study, including raw data and source data underlying figures, has been deposited in Figshare https://doi.org/10.6084/m9.figshare.25493419 . Mean annual temperature at each site was extracted from the WorldClim database https://www.worldclim.org/ . Soil cation exchange capacity, total nitrogen, pH, bulk density, organic carbon density and sand content were extracted from Soil Grid database https://files.isric.org/soilgrids/latest/data_aggregated/1000m/ . Soil water content was obtained from ERA5-Land database https://www.ecmwf.int/en/era5-land . Global map was downloaded from natural earth https://www.naturalearthdata.com/ . Source data are provided with this paper.
Code availability
All R code for reproducing the mainly results are available at Figshare https://doi.org/10.6084/m9.figshare.25493419 .
Rockstroem, J. et al. Safe and just Earth system boundaries. Nature 619 , 102–111 (2023).
Article ADS CAS Google Scholar
Seddon, N. et al. Understanding the value and limits of nature-based solutions to climate change and other global challenges. Philos. Trans. R. Soc. B-Biol. Sci. 375 , 20190120 (2020).
Article Google Scholar
Griscom, B. W. et al. Natural climate solutions. Proc. Natl Acad. Sci. USA 114 , 11645–11650 (2017).
Article ADS CAS PubMed PubMed Central Google Scholar
Anderegg, W. et al. Climate-driven risks to the climate mitigation potential of forests. Science 368 , eaaz7005 (2020).
Article CAS PubMed Google Scholar
Li, H. et al. Nitrogen addition delays the emergence of an aridity-induced threshold for plant biomass. Natl. Sci. Rev. 10 , nwad242 (2023).
Article PubMed PubMed Central Google Scholar
Seabloom, E. W. et al. Increasing effects of chronic nutrient enrichment on plant diversity loss and ecosystem productivity over time. Ecology 102 , e03218 (2021).
Article PubMed Google Scholar
Eskelinen, A., Harpole, W. S., Jessen, M.-T., Virtanen, R. & Hautier, Y. Light competition drives herbivore and nutrient effects on plant diversity. Nature 611 , 301–305 (2022).
Hautier, Y., Niklaus, P. A. & Hector, A. Competition for light causes plant biodiversity loss after eutrophication. Science 324 , 636–638 (2009).
Article ADS CAS PubMed Google Scholar
Harpole, W. S. et al. Addition of multiple limiting resources reduces grassland diversity. Nature 537 , 93–96 (2016).
Band, N., Kadmon, R., Mandel, M. & DeMalach, N. Assessing the roles of nitrogen, biomass, and niche dimensionality as drivers of species loss in grassland communities. Proc. Natl Acad. Sci. USA 119 , e2112010119 (2022).
Article CAS PubMed PubMed Central Google Scholar
Tilman, D. Resource Competition and Community Structure (Princeton University Press, 1982).
Newman, E. I. Competition and diversity in herbaceous vegetation. Nature 244 , 310 (1973).
Article ADS Google Scholar
Harpole, W. S. & Tilman, D. Grassland species loss resulting from reduced niche dimension. Nature 446 , 791–793 (2007).
Farrer, E. C. & Suding, K. N. Teasing apart plant community responses to N enrichment: the roles of resource limitation, competition and soil microbes. Ecol. Lett. 19 , 1287–1296 (2016).
Stevens, C. J. et al. Nitrogen deposition threatens species richness of grasslands across Europe. Environ. Pollut. 158 , 2940–2945 (2010).
Crawley, M. J. et al. Determinants of species richness in the park grass experiment. Am. Nat. 165 , 179–192 (2005).
Britto, D. T. & Kronzucker, H. J. NH 4 + toxicity in higher plants: a critical review. J. Plant Physiol. 159 , 567–584 (2002).
Article CAS Google Scholar
Zhu, J., Zhang, Y., Yang, X., Chen, N. & Jiang, L. Synergistic effects of nitrogen and CO 2 enrichment on alpine grassland biomass and community structure. N. Phytol. 228 , 1283–1294 (2020).
De Melo, T. R. et al. Biogenic aggregation intensifies soil improvement caused by manures. Soil Tillage Res. 190 , 186–193 (2019).
Cai, A. et al. Manure acts as a better fertilizer for increasing crop yields than synthetic fertilizer does by improving soil fertility. Soil Tillage Res. 189 , 168–175 (2019).
Tian, Q. et al. A novel soil manganese mechanism drives plant species loss with increased nitrogen deposition in a temperate steppe. Ecology 97 , 65–74 (2016).
Ryals, R., Eviner, V. T., Stein, C., Suding, K. N. & Silver, W. L. Grassland compost amendments increase plant production without changing plant communities. Ecosphere 7 , e01270 (2016).
Verma, P. et al. Variations in soil properties, species composition, diversity, and biomass of herbaceous species due to ruminant dung residue in a seasonally dry tropical environment of India. Trop. Grassl. -Forrajes Trop. 3 , 112–128 (2015).
Critchley, C. N. R. et al. Plant species richness, functional type and soil properties of grasslands and allied vegetation in English environmentally sensitive areas. Grass Forage Sci. 57 , 82–92 (2002).
Schmid, B. The species richness-productivity controversy. Trends Ecol. Evol. 17 , 113–114 (2002).
Stockmann, U. et al. The knowns, known unknowns and unknowns of sequestration of soil organic carbon. Agric. Ecosyst. Environ. 164 , 80–99 (2013).
Jobbágy, E. G. & Jackson, R. B. The vertical distribution of soil organic carbon and its relation to climate and vegetation. Ecol. Appl. 10 , 423–436 (2000).
Brahim, N. et al. Soil OC and N stocks in the saline soil of tunisian gataaya oasis eight years after application of manure and compost. Land 11, 442 (2022).
Keller, A. B. B. et al. Stronger fertilization effects on aboveground versus belowground plant properties across nine US grasslands. Ecology 104 , e3891 (2023).
Crowther, T. W. et al. Sensitivity of global soil carbon stocks to combined nutrient enrichment. Ecol. Lett. 22 , 936–945 (2019).
Doetterl, S. et al. Soil carbon storage controlled by interactions between geochemistry and climate. Nat. Geosci. 8 , 780–783 (2015).
Nuralykyzy, B. et al. Influence of land use types on soil carbon fractions in the Qaidam Basin of the Qinghai-Tibet Plateau. Catena 231 , 107273 (2023).
Wieder, W. R., Cleveland, C. C., Smith, W. K. & Todd-Brown, K. Future productivity and carbon storage limited by terrestrial nutrient availability. Nat. Geosci. 8 , 441–444 (2015).
Bai, Y. & Cotrufo, M. F. Grassland soil carbon sequestration: current understanding, challenges, and solutions. Science 377 , 603–608 (2022).
Gross, A. & Glaser, B. Meta-analysis on how manure application changes soil organic carbon storage. Sci. Rep. 11 , 5516 (2021).
Toth, G., Jones, A. & Montanarella, L. The LUCAS topsoil database and derived information on the regional variability of cropland topsoil properties in the European Union. Environ. Monit. Assess. 185 , 7409–7425 (2013).
Liu, Y. et al. Meta-analysis on the effects of types and levels of N, P, and K fertilization on organic carbon in cropland soils. Geoderma 437 , 116580 (2023).
Kan, Z.-R. et al. Mechanisms of soil organic carbon stability and its response to no-till: a global synthesis and perspective. Glob. Change Biol. 28 , 693–710 (2022).
White, R. P., Murray, S., Rohweder, M., Prince, S. & Thompson, K. Grassland ecosystems . (World Resources Institute Washington, DC, USA, 2000).
Ma, J. et al. Assessing the impacts of agricultural managements on soil carbon stocks, nitrogen loss, and crop production-a modelling study in eastern Africa. Biogeosciences 19 , 2145–2169 (2022).
A, Y. et al. Vertical variations of soil water and its controlling factors based on the structural equation model in a semi-arid grassland. Sci. Total Environ. 691 , 1016–1026 (2019).
Zhang, Y. et al. Multi-decadal trends in global terrestrial evapotranspiration and its components. Sci. Rep. 6 , 1–12 (2016).
Google Scholar
Kidd, J., Manning, P., Simkin, J., Peacock, S. & Stockdale, E. Impacts of 120 years of fertilizer addition on a temperate grassland ecosystem. PLoS One 12 , e0174632 (2017).
Rawls, W. J., Pachepsky, Y. A., Ritchie, J. C., Sobecki, T. M. & Bloodworth, H. Effect of soil organic carbon on soil water retention. Geoderma 116 , 61–76 (2003).
Zheng, Y. et al. Determining the harvest frequency to maintain grassland productivity and minimum nutrient removal from soil. Plant Soil 487 , 79–91 (2023).
Zhang, L. et al. Habitat heterogeneity induced by pyrogenic organic matter in wildfire-perturbed soils mediates bacterial community assembly processes. ISME J. 15 , 1943–1955 (2021).
CatenaElser, J. J. et al. Global analysis of nitrogen and phosphorus limitation of primary producers in freshwater, marine and terrestrial ecosystems. Ecol. Lett. 10 , 1135–1142 (2007).
Wassen, M. J., Venterink, H. O., Lapshina, E. D. & Tanneberger, F. Endangered plants persist under phosphorus limitation. Nature 437 , 547–550 (2005).
Venterink, H. O., Wassen, M. J., Verkroost, A. W. M. & de Ruiter, P. C. Species richness-productivity patterns differ between N-, P-, and K-limited wetlands. Ecology 84 , 2191–2199 (2003).
Gautam, A. et al. Responses of soil microbial community structure and enzymatic activities to long-term application of mineral fertilizer and beef manure. Environ. Sustain. Indic. 8 , 100073 (2020).
Du, Y. et al. Effects of manure fertilizer on crop yield and soil properties in China: a meta-analysis. Catena 193 , 104617 (2020).
Li, S. X., Wang, Z. H., Hu, T. T., Gao, Y. J. & Stewart, B. A. in Advances in Agronomy Vol. 101 (ed Donald L. Sparks) 123–181 (Academic Press, 2009).
Naeem, M., Ansari, A. & Gill, S. Contaminants in Agriculture: Sources, Impacts and Management (Springer International Publishing, Cham, Switzerland, 2020).
Rambaut, L.-A. E., Tillard, E., Vayssieres, J., Lecomte, P. & Salgado, P. Trade-off between short and long-term effects of mineral, organic or mixed mineral-organic fertilisation on grass yield of tropical permanent grassland. Eur. J. Agron . 141 , 126635 (2022).
Stevens, H. & Carson, W. Resource quantity, not resource heterogeneity, maintains plant diversity. Ecol. Lett. 5 , 420–426 (2002).
Taddesse, G., Peden, D., Abiye, A. & Wagnew, A. Effect of manure on grazing lands in Ethiopia, East African highlands. Mt. Res. Dev. 23 , 156–160 (2003).
Loydi, A. & Martin Zalba, S. Feral horses dung piles as potential invasion windows for alien plant species in natural grasslands. Plant Ecol. 201 , 471–480 (2009).
Ye, J.-S., Bradford, M. A., Maestre, F. T., Li, F.-M. & Garcia-Palacios, P. Compensatory thermal adaptation of soil microbial respiration rates in global croplands. Glob. Biogeochem. Cycle 34 , e2019GB006507 (2020).
Ye, J.-S., Bradford, M. A., Dacal, M., Maestre, F. T. & Garcia-Palacios, P. Increasing microbial carbon use efficiency with warming predicts soil heterotrophic respiration globally. Glob. Change Biol. 25 , 3354–3364 (2019).
Li, F. et al. Warming alters surface soil organic matter composition despite unchanged carbon stocks in a Tibetan permafrost ecosystem. Funct. Ecol. 34 , 911–922 (2020).
Chen, Y., Feng, J., Yuan, X. & Zhu, B. Effects of warming on carbon and nitrogen cycling in alpine grassland ecosystems on the Tibetan Plateau: a meta-analysis. Geoderma 370 , 114363 (2020).
Yan, Z. et al. Changes in soil organic carbon stocks from reducing irrigation can be offset fertilizer in the North China Plain. Agric. Water Manag. 266 , 107539 (2022).
Gaudare, U. et al. Soil organic carbon stocks potentially at risk of decline with organic farming expansion. Nat. Clim. Change 13 , 719–725 (2023).
Rui, Y. et al. Persistent soil carbon enhanced in Mollisols by well- managed grasslands but not annual grain or dairy forage cropping systems. Proc. Natl Acad. Sci. USA 119 , e2118931119 (2022).
Kallenbach, C. & Grandy, A. S. Controls over soil microbial biomass responses to carbon amendments in agricultural systems: ameta-analysis. Agric. Ecosyst. Environ. 144 , 241–252 (2011).
Hedges, L. V., Gurevitch, J. & Curtis, P. S. The meta-analysis of response ratios in experimental ecology. Ecology 80 , 1150–1156 (1999).
Murphy, G. E. P. & Romanuk, T. N. A meta-analysis of declines in local species richness from human disturbances. Ecol. Evol. 4 , 91–103 (2014).
Lajeunesse, M. J. On the meta-analysis of response ratios for studies with correlated and multi-group designs. Ecology 92 , 2049–2055 (2011).
Song, C., Peacor, S. D., Osenberg, C. W. & Bence, J. R. An assessment of statistical methods for nonindependent data in ecological meta-analyses. Ecology 101 , e03184 (2020).
Viechtbauer, W. Conducting meta-analyses in R with the metafor package. J. Stat. Softw. 36 , 1–48 (2010).
Egger, M., Smith, G. D., Schneider, M. & Minder, C. Bias in meta-analysis detected by a simple, graphical test. BMJ-Br. Med. J. 315 , 629–634 (1997).
Fick, S. E. & Hijmans, R. J. WorldClim 2: new 1-km spatial resolution climate surfaces for global land areas. Int. J. Climatol. 37 , 4302–4315 (2017).
Hengl, T. et al. SoilGrids1km-Global Soil Information Based on Automated Mapping. PLoS One 9 , e105992 (2014).
Article ADS PubMed PubMed Central Google Scholar
Munoz-Sabater, J. et al. ERA5-Land: a state-of-the-art global reanalysis dataset for land applications. Earth Syst. Sci. Data 13 , 4349–4383 (2021).
Schermelleh-Engel, K., Moosbrugger, H. & Müller, H. Evaluating the fit of structural equation models: tests of significance and descriptive goodness-of-fit measures. Methods Psychol. Res. Online 8 , 23–74 (2003).
Warton, D. I., Wright, I. J., Falster, D. S. & Westoby, M. Bivariate line-fitting methods for allometry. Biol. Rev. 81 , 259–291 (2006).
Download references
Acknowledgements
This research was supported by the Fundamental Research Funds for the Central Universities (lzujbky-2022-ct01) and “111” Project (BP0719040). S.L.C. was supported by NSF award DEB-1856383. J.P. and J.S. were supported by the Spanish Government grants PID2020115770RB-I, PID2022-140808NB-I00, and TED2021-132627 B–I00 funded by MCIN, AEI/10.13039/ 501100011033 European Union Next Generation EU/PRTR, the Fundación Ramón Areces grant CIVP20A6621, and the Catalan Government grants SGR 2021–1333 and AGAUR2023 CLIMA 00118. We thank Prof. Chao Song for suggestions and comments on the writing.
Author information
Authors and affiliations.
State Key Laboratory of Herbage Improvement and Grassland Agro-ecosystems, College of Ecology, Lanzhou University, Lanzhou, 730000, China
Ting-Shuai Shi, Hailing Li & Jian-Sheng Ye
Department of Biology, University of New Mexico, Albuquerque, NM, 87131, USA
Scott L. Collins
High Meadows Environmental Institute, Princeton University, Princeton, NJ, USA
Kailiang Yu
CSIC, Global Ecology Unit CREAF-CSIC-UAB, Barcelona, 08193, Spain
Josep Peñuelas & Jordi Sardans
CREAF, Cerdanyola del Vallès, Barcelona, 08193, Spain
You can also search for this author in PubMed Google Scholar
Contributions
J.-S.Ye. and T.-S.Shi. conceived this study. T.-S.Shi. and H.-L.Li. collected data. T.-S.Shi. performed the analysis. J.-S.Ye. and T.-S.Shi. drafted the paper. S.L.Collins., K.Yu., J.Peñuelas. and J.Sardans. wrote and edited the final version of the manuscript. All authors contributed critically to the writing and revising of the manuscript.
Corresponding author
Correspondence to Jian-Sheng Ye .
Ethics declarations
Competing interests.
The authors declare no competing interests.
Peer review
Peer review information.
Nature Communications thanks the anonymous reviewers for their contribution to the peer review of this work. A peer review file is available.
Additional information
Publisher’s note Springer Nature remains neutral with regard to jurisdictional claims in published maps and institutional affiliations.
Supplementary information
Supplementary information, reporting summary, description of additional supplementary files, peer review file, supplementary data 1, source data, source data, rights and permissions.
Open Access This article is licensed under a Creative Commons Attribution 4.0 International License, which permits use, sharing, adaptation, distribution and reproduction in any medium or format, as long as you give appropriate credit to the original author(s) and the source, provide a link to the Creative Commons licence, and indicate if changes were made. The images or other third party material in this article are included in the article’s Creative Commons licence, unless indicated otherwise in a credit line to the material. If material is not included in the article’s Creative Commons licence and your intended use is not permitted by statutory regulation or exceeds the permitted use, you will need to obtain permission directly from the copyright holder. To view a copy of this licence, visit http://creativecommons.org/licenses/by/4.0/ .
Reprints and permissions
About this article
Cite this article.
Shi, TS., Collins, S.L., Yu, K. et al. A global meta-analysis on the effects of organic and inorganic fertilization on grasslands and croplands. Nat Commun 15 , 3411 (2024). https://doi.org/10.1038/s41467-024-47829-w
Download citation
Received : 12 October 2023
Accepted : 15 April 2024
Published : 22 April 2024
DOI : https://doi.org/10.1038/s41467-024-47829-w
Share this article
Anyone you share the following link with will be able to read this content:
Sorry, a shareable link is not currently available for this article.
Provided by the Springer Nature SharedIt content-sharing initiative
This article is cited by
Soil water regulates plant diversity response to gradual and step nitrogen addition.
- Jiu-Ying Pei
- Jian-Sheng Ye
Plant and Soil (2024)
Quick links
- Explore articles by subject
- Guide to authors
- Editorial policies
Sign up for the Nature Briefing newsletter — what matters in science, free to your inbox daily.



IMAGES
VIDEO
COMMENTS
Join us on a weeks-long journey through a home gardener's tomato fertilizer experiment! She has taken on the challenge of testing three different types of fertilizers - both inorganic and organic - to determine which one would work best for her precious tomato plants. The three fertilizers in question are Miracle Grow Tomato and Vegetable ...
However, there are different kinds of fertilizer, and some believe that fertilizer is simply uncessary. In this experiment, we'll compare two different kinds of fertilizer to normal soil. Materials: Chemical fertilizer, such as Miracle-Gro; Organic fertilizer (pelleted chicken manure, bone meal, etc.) Seeds (of any kind, but keep it at the same ...
Inorganic fertilizers also don't require as much pH management. In an experiment comparing an inorganic fertilizer with an organic fertilizer applied to hydroponically grown lettuce, the organic fertilizer caused wild pH swings. The pH often increased above 8, making nutrients unavailable to the plants.
Chemical Fertilizer vs Organic Fertilizer Science Assessment Task I became interested in this project because I wanted to know which fertilizer makes plants grow the fastest. Research I found background information that was very useful towards my experiment. I found out that
The purpose of this experiment is to find out which type of fertilizer, whether organic or inorganic, speeds the growth of plants. Additional information The hypothesis is that organic fertilizer will speed the growth of the plants due to the lack of toxins and pesticides found within the fertilizer.
In this study, we calculated two types of response ratio: (1) organic, inorganic fertilization relative to ambient condition (i.e., Org vs. Amb, Inorg vs. Amb) of all experiments, and (2) organic ...
Organic agriculture science fair projects and experiments: topics, ideas, resources, and sample projects. Organic Agriculture ... Determine the effect of organic fertilizer and natural farming soils (clay soil and sandy loam) on corn plants. ... Improved Rooting & Companion Planting How fertilizers, inorganic and organic, affect Eisenia fetida ...
p>A Greenhouse experiment was conducted to identify the suitable dose of organic fertilizer for lettuce production. Different doses of organic fertilizer (6.5, 13 and 26 t ha<sup>-1</sup>) and the ...
Experiments were conducted to compare the effects of organic and synthetic fertilizers on soil fertility and wheat (Triticum aestivum L.) productivity. Soil fertility and protein contents of wheat grains (13.2% and 13.3% during 2005-06 and 2006-07, respectively) were improved by organic amendments.
It doesn't, however, contain any potassium (which is one of the most important plant nutrients) so it often needs to be mixed with sulphate of potash. Blood & Bone is a by-product of the meat works so is completely organic. It contains dried blood, bone dust and meat waste. The yates Gro-plus fertilizer I used contains 6% nitrogen and 7% ...